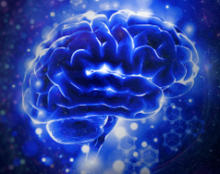
Demonstration of the feasibility of using a fiberless optoelectrode for activating and recording from neurons… New insights into the mechanisms of neurovascular coupling… Chronic in vivo recordings with carbon fiber microelectrodes… In vivo subcellular imaging of voltage and calcium signals…
Newly designed fiberless multi-color optoelectrode for in vivo use
Optogenetics is a promising technology that offers the opportunity to probe the function of the brain at an unprecedented level of cellular and functional specificity. Using different forms of light-sensitive genetic material, it has become possible to target specific cell types within a given region of the brain for modulation. Based on the opsin that is inserted and the wave length of the light used, one has the ability to temporarily activate or inactivate the targeted cell type. As a relatively new technique, optogenetics’ applications are advancing at an accelerated pace, with frequent, elegant solutions to technical challenges. BRAIN awardee Dr. Euisik Yoon and colleagues at the University of Michigan recently published an article in Scientific Reports that details a number of technical advances to their novel optoelectrode. The new electrode uses a combination of injection laser diode (ILD) and gradient-index (GRIN) lenses to achieve a fiberless system that can deliver multiple wavelengths of light to the same brain area. Additionally, this novel recording system protects both tissue and electrode from electrical and thermal damage. As a proof of principle, the researchers demonstrated they could simultaneously record neural activity from the electrodes while stimulating with two different wavelengths of light to both enhance and inhibit neural activity in hippocampal CA1 pyramidal cells in anesthetized mice. This new neural modulatory and recording technology represents an impressive advancement in the field of optogenetics, which will likely lead to new research opportunities and a better understanding of neural network dynamics.
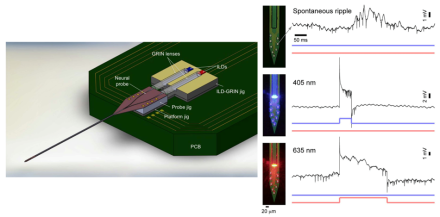
Cell type-specific contributions to the BOLD signal
Understanding the neural underpinnings of the change in blood flow and volume due to neural activity, the hemodynamic response, is critical to fully interpret the findings from fMRI experiments. As such, the study of the relationship between local neural activity and subsequent changes in cerebral blood flow, known as neurovascular coupling, and its association with particular cell types is an important part of the BRAIN Initiative®. BRAIN grantee Dr. Anna Devor and her team recently published an article in eLIfe that used a combination of neural recording techniques to determine which cells are actively involved in neurovascular coupling. Using optogenetic modulation and recordings of local neuronal activity with two-photon imaging in mice, the group identified particular mechanisms responsible for vasoconstriction, which is a main component of the blood oxygenation level dependent (BOLD) fMRI signal. Specifically, they found that inhibitory neurons in the cerebral cortex are responsible for vasoconstriction through the release of a signaling molecule, neuropeptide Y (NPY), which acts on NPY-Y1 receptors located on blood vessels in the brain. Excitatory neurons were not involved in vasoconstriction; however, stimulation of either inhibitory or excitatory neurons could lead to vasodilation. To compare the optogenetic stimulation to normal sensory conditions, the researchers recorded from the same region of the somatosensory cortex during forepaw stimulation and optogenetic modulation, stimulation of inhibitory neurons produced a similar effect to sensory stimulation within the same neural tissue. The results of this study shed light on which cell types are responsible for particular components of the BOLD response, and are likely to have an important impact on the interpretation of fMRI studies across species.
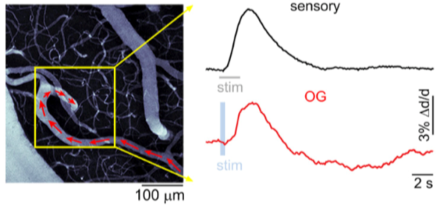
New carbon fiber electrodes show long-term stability with minimal damage to the brain
One of the goals of the NIH BRAIN Initiative is to develop and apply improved methods for large-scale monitoring of neural activity. Chronic electrophysiological recordings in animal studies, as well as in human studies such as those involving deep brain stimulation and brain machine interface systems, are limited by current electrode technology. NIH BRAIN awardee Dr. Cynthia Chestek and her colleagues from the University of Michigan and the University of Pittsburgh recently published a paper in the Journal of Neural Engineering that assessed a new type of microelectrode array for long-term recordings. To reduce inflammation caused by typical electrodes, Chestek and colleagues have developed a microelectrode that is one-fifth the size of a human hair in diameter, or approximately eight microns. Previously demonstrated to record high quality neural activity, the electrodes are made of carbon fiber, insulated with parylene-c, and the end sites are coated with poly(3,4-ethylenedioxythiophene) (PEDOT), a conductive material. Chestek and her team showed that when chronically implanted, these electrode arrays detected neural activity for at least three months in the motor cortex of rats. Further, these microelectrodes produced significantly less damage to the brain, as measured by glial scarring and neuronal cell counts, compared to commercially available silicon electrodes. Indeed, these carbon fiber arrays have the potential to greatly improve long-term, in vivo recordings for measuring dynamic activity within the brain.
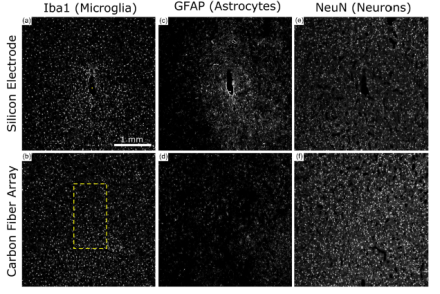
New insights into in vivo neural processing using voltage and calcium imaging
Determining how neural information is processed across synapses in vivo has been a challenge for neuroscientists. In a recent publication in Cell, BRAIN grantee Dr. Thomas Clandinin from Stanford University and colleagues provided important insight into the dynamics of in vivo neural processing. The authors used two-photon imaging of genetically-encoded voltage and calcium indicators to study synaptically-connected axons and dendrites in the visual system of Drosophila. First, the team validated a new voltage indicator, ASAP2f. Specifically, ASAP2f was expressed in individually identifiable neurons in the optic lobe and visually-evoked responses were measured using two-photon microscopy in the awake fly. The team demonstrated that the temporal features of this indicator in response to light were similar to previously recorded electrophysiological responses from these neurons, suggesting that the voltage imaging was an accurate readout of the biological response. Next, the researchers compared subcellular imaging of voltage and calcium signals in the fly optic lobe in response to the same light stimuli. Unlike voltage signals, calcium signaling patterns varied across subcellular compartments in the same cell, with that pattern remaining similar for cells of the same cell type. These results demonstrate the need to cautiously interpret findings based on different types of indicators, as well as the importance of using more than one indicator to provide a more complete representation of information flow between neurons.
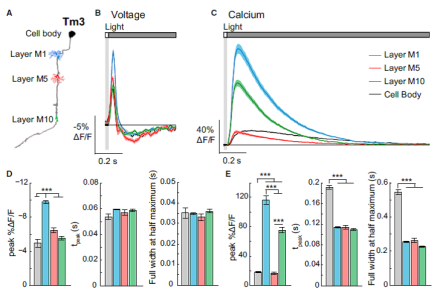